Introduction
During the planning, designing, and construction stages of urban infrastructure, territorial authorities must consider contingency measures in order to reduce the environmental impact of storm runoff. Such measures are aimed at improving the management of drainage water, including the estimation of its impact (Grenestam and Nordin, 2018). Stormwater treatment has become an essential component in urban and suburban areas to manage stormwater runoff (Yang et al., 2013). Although a conventional way of treating urban flooding is constructing sewage systems, lagoons, and artificial wetlands, an alternative approach has to be developed because of their significant cost and large area requirements, especially in tropical Latin American cities, characterized by accelerated demographic growth rates and little planned infrastructure. This triggers serious alterations in the urban environment such as loss of vegetation, reduced plant cover deepening the impacts of storms, increasing flood frequency and the discharge of chemicals, and excess of nutrients in aquatic ecosystems. Thus, it has resulted in general eutrophication and diminishing the ecological and environmental quality of aquatic ecosystems (Warn and Adamo, 2014; Wilkinson, 2017).
One of the ways that tropical cities can reduce the impact of runoff on their aquatic ecosystems is through the implementation of rain gardens, which have recently been recommended as the best management practice for the treatment of stormwater runoff in temperate zones such as in northern Europe and America, where the demand has increased in recent years (Church, 2015; Dietz and Clausen, 2006; Muthanna et al., 2008). They are attractive given certain advantages: the technology occupies little area, is of low cost, significantly decreases runoff, and improves the water quality in a natural way; the rain gardens are aesthetically beautiful and help improve the landscape since they can be built in front and backyards, and even in commercial areas (Dussaillant et al., 2004; Ishimatsu et al., 2017; Muthanna et al., 2007; Rodríguez, 2014).
Thus, rain gardens could generate important benefits in the tropical zones, especially in those where there is a marked seasonality with periods of rain and drought. This type of technology is highly beneficial since the soil of these gardens absorb and store rainwater, which allows plants to grow and helps remove a large part of the nutrients and pollutants from runoff. Moreover, the environmental impact on the aquatic ecosystems would be significantly reduced, as well as this contributes to the embellishment of the landscape, which would help sustain the biodiversity of native plants and animals associated with these in urban areas (Hostetler, 2009). This sustainable economic alternative would help recover the ecosystem and provide services such as regulation of atmospheric gases and the diminishing of runoff volumes, of the fixation of pathogenic microorganisms and pollutants such as heavy metals and hydrocarbons, and of nutrients such as phosphorus, potassium and nitrogen (Yang et al., 2010).
Accordingly, the present study focuses on evaluating the elimination of nitrogen compounds using a pilot rain garden as an alternative method to reduce nutrients and contaminants present in the runoff of stormwater in cities of the Caribbean region in Colombia.
Methodology
The rain garden constructed in this investigation includes several components of diverse nature that work together to remove the nitrogen present in the runoff water. The experimental considerations followed during August 2016, under which the functioning of the garden was evaluated, are described below.
Construction of the rain garden
The prototype of the rain garden was constructed at Piedra de Bolívar campus from Universidad de Cartagena, following instructions from Urban Drainage and Flood Control District (2010), on the surface of the ground; therefore, its walls were made in concrete of 3 000 psi (lb/in2). This should have been waterproofed inside; this system had a total capacity of 6.75 m3. Inside it had four layers of soil, between each of them a geotextile was placed to not allow the exchange of material between one layer and another. In the upper layer were planted plants of Duranta golden; this species can withstand long periods of drought. Besides being a species of low economic cost and easy acquisition, it also allows beautifying the garden (Suekane et al., 2012).
The outlet of the rain garden was constructed using a PVC tube with a diameter of 0.11 m and 3.5 m, a longitudinal slope of 2 %, and density of a performed area of 1.03 cm2/m (Figure 1). The area of bio-retention and the depth of ponding (0.2 m) being known, the volume of storage of the system was obtained using the method of continuity of masses (Equation 1), giving a volume of 0.765 m3.
Where:
As: is the bio-retention area
ds: is the depth of ponding
Fv: is the fraction of the area occupied by vegetation
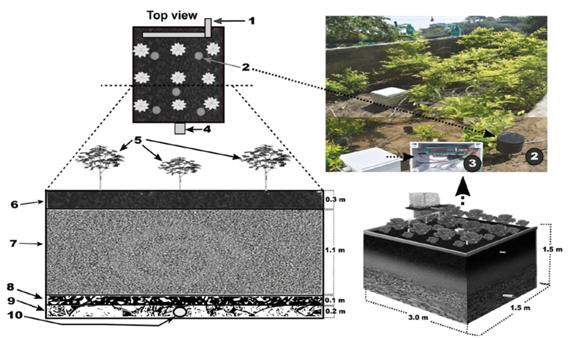
Figure 1 Rain garden components: 1. Affluent; 2. Automatic monitoring sensors (pH, humidity, and temperature); 3. An Arduino sensor for collected data processing; 4. Effluent; 5. Duranta golden specimens (density: 5 specimens/m2); 6. Organic fertilizer superficial layer; 7. Sand layer (0.075 mm - 0.5 mm); 8. Fine gravel layer (5-20 mm); 9. Coarse gravel layer (20-80 mm); 10. Outlet pipe.
Experimental design
To conduct this research, an experimental design of unifactorial type was followed (Montgomery 2004), having the runoff rate as the main factor with eight treatments including control (Table 1), having as a response variable the rate of nitrogenous compound removal, whose description will be given later. Each treatment consisted in the simulation of a singling event with different discharge value (0.13 L/min/m2, 0.32 L/min/m2, 0.58 L/min/m2, 0.91 L/min/m2, 1.85 L/min/m2, 2.14 3.38 L/min/m2, and 4.04 L/min/m2), using a solution with 25 mg/L of nitrogen (except control), superior to those reported for runoff in undeveloped basins (Dietz and Clausen, 2005). This solution was prepared with a commercial fertilizer (Urea: 46 % of N). The keep the flow of the content constant, it was modified with the Mariotte system, also controlling the outflow with a stopcock.
Each experiment was performed by duplicate, every two days and at the same time. The physicochemical variables of the water, pH, and temperature were measured in a sample of water collected in an 18 L bucket, with an OHAUS Starter 300 pH meter (measuring range: pH: 0-14 and temperature: 0-100 °C), while the conductivity was measured using an OHAUS Starter 300 conductivity meter (measuring range: 0-199.9 mS/cm). In the case of the soil, the variables pH, humidity (%), and temperature (ºC) were automatically and directly monitored by sensors connected to an Arduino platform PC located in the garden (Figure 1).
Nitrogen removal
To calculate the rate of nitrogen removal in the rain garden, we compared the ingoing and outgoing amounts of those nitrogen compounds that are usually found in natural systems (nitrites, nitrates, TKN, ammonia, and total nitrogen), five samples of 500 g from soil were randomly collected at the beginning and at the end of each experiment, while the water samples (1L) were taken in the inlet and outlet of this rain garden packed and stored at 4 °C for later analysis. The nitrogen compound analysis was performed at the environmental quality government laboratory of the Corporación Regional Autónoma del Canal del Dique (CARDIQUE), using a colorimetric technique with a Varian Agilent Cary 100 spectrophotometer, following the standard methods 4 500 B.C. (Rice et al., 2017). The kinetics of nutrient removal was calculated according to Equation 2.
Where
%R: Removal percentage
C 0 : Concentration of analyte in the inlet.
C f : Concentration of analyte in the outlet.
Data analysis
Data were stored in Microsoft Office Excel spreadsheets and subsequently analyzed with SPSS 21 and Prism 6.0 statistical packages. An initial descriptive analysis was performed including the computation of arithmetic means and standard deviations. These values were compared using the one-way ANOVA inferential technique while the differences between the inlet and outlet values were analyzed by a t-Student test. Prior to each analysis, the assumptions of normality, homoscedasticity, and data independence were verified by non-parametric methods in cases where the assumptions were not met. In all cases, the level of significance (p-value) was 0.05.
Results
Physicochemical parameters of soil and water
The experimental assays of the runoff simulation allowed determining that, despite maintaining a constant flow rate, the mean retention time in the system varied between 8.00 and 14.16 minutes for the different treatments (T), being as indicated below: T4: 8.02 < T3:8.18 < T1:9.1 < Control:9.26 < T5,T6: 10.01 < T7:14.16.
Regarding the soil temperature for the different experiment, this is shown in Figure 2a. The values oscillate between 30 °C and 33 °C, without presenting statistically significant differences (p-value > 0.05). In the case of soil humidity, Figure 2b shows that the high humidity values occur when the runoff flows are equal or less than 0.98 L/min/m2. Concerning the soil, the pH remained constant across almost all of the runoff simulation events (Figure 2c), except for the lowest discharger in which the pH was significantly lower than the others (p-value < 0.005; mean 7.8).
The water temperature average behavior is shown in Figure 2d, where it can be observed that there are no statistically significant differences (p-value > 0.005) between the temperature values of the inlet and outlet. On the other hand, the water conductivity values were significantly higher (p-value < 0.05) for the outlet than the inlet for all experiments (Figure 2e). The water pH varied between 7.8 and 8.0, also presenting statistically significant differences (p-values < 0.05) between the inlet and the outlet (Figure 2f).
Variation of nitrogenous compounds in soil and water
Five nitrogenous compounds were analyzed in the soil; the results are shown in Figure 3a-e. There were no statistically significant differences (p-value > 0.05) between the values of the analyzed compounds at the beginning and end of the runoff simulations, except for the ammonium concentration which decreased significantly (p-value < 0.05).
The results for the nitrogen compounds analyzed in the water are shown in Figure 3f-h. There is no retention of the total nitrogen when the runoff volume hitting the rain garden is equal or less than 0.32 L/min/m2. In the case of the total Kjeldahl nitrogen (TKN), the system showed a reduction for almost all of the simulated runoff volume rates, except for those where the runoff volume values were 0.91 and 1.85L/min/m2. The above results indicate that, except for the nitrate, the rain garden system is capable of retaining more of the nitrogen compounds, especially when the runoff volume values are equal or less than 0.32 L/min/m2.
Concentrations of nutrients analyzed in affluent and effluent in the rain garden are shown in Figure 3. The total nitrogen was significantly reduced (p-values < 0.05). For the two lowest flow rates, there was no effect for the third one, and, after that, an inverse effect occurs (Figure 3f). On the other hand, the nitrate concentration increased significantly and progressively in the effluent after each experiment (Figure 3g), showing that nitrate mobility increases when the prepared nitrogen solution enters the system. Conversely, the TKN concentration increased in the outlet only when the runoff rates were 0.91 L/min/m2 or 1.85 L/min/m2 (Figure 3h).
Discussion
Physicochemical parameters
The results of soil temperature can be explained by the presence of vegetal cover reducing the solar incidence in the system and, therefore, reducing the evaporation rate of water, nutrients, and some volatile compounds present in the soil, also allowing the stability of biochemical processes occurring in this soil matrix by nitrogen-fixing microorganism like nitrifier bacterias, which help to improve performance of rain gardens (Özkan and Gökbulak, 2017; Hong et al., 2018).
Regarding the results of soil humidity, it is important to note that abundant water retention occurs when the volume of water poured in the soil cannot overcome the gravity force and drain freely against capillary and electrostatic action of the particles in the soil (Shukla, 2013). Contrary to expectations, when the volume of water poured is equal or greater than 1.85 L/min/m2, the humidity water drains freely, and the humidity is not at the maximum rate. Authors such as Dong and Horton (1993) determined the infiltration capacity of the soil as an exponential function of the time that decreases until reaching a constant rate. However, other authors consider that the water infiltration in the soil also depends on aspects such as the granulometric profile, the microorganisms composition, and the presence of layers of vegetation and invertebrates (Bonin et al., 2012; Mailapalli et al., 2013).
Results indicate that the pH was stable throughout the experimental period. This stability is explained by the pH buffering capacity that maintains the necessary conditions for the development of the biochemical processes. These processes can be the nitrogen mineralization capacity and the absorption rate of NH4+; they are related to the increase in pH (Black, 1975) that is maximized when the value of this is close to 8.0 (Aye et al., 2018). Additionally, the pH stability favors the growth of plants and edaphic wildlife that both play a determinant role in the absorption of nitrogen compounds such as ammonia (NH4 +) (Fan et al., 2018; Mehring et al., 2016).
In relation to the water physicochemical variables, the temperature indicates that the system had a low rate of soil/water heat exchange (low enthalpy), withstanding the stability of the biochemical processes between plants and poikilotherm animals and recognizing the nutrient fixation of great importance in this type of system. The opposite occurred with the conductivity, which was greater in the effluent, indicating that the ion’s concentration increases due to the soil leaching (Raij et al., 2018). Although this behavior of the conductivity values was expected, it must be taken into account that the high values of this parameter in water could negatively affect the water quality; however, this drawback can be corrected by increasing the density of plants, especially on the banks of aquatic ecosystems (Rodrigues et al., 2018). Furthermore, the differences in pH values between the inlet and the outlet are in progressive increase in the outlet. These differences can be explained by the generation of chemical species, such as the anion bicarbonate (HCO3 -) and ammonium (NH4), from the hydrolysis of the urea that was incorporated to the systems, which, according to Lei et al. (2018), is directly related to the increase of the soil pH. However, these pH values do not represent risks for most aquatic species, especially those that make calcium carbonate skeletons or shells (Bednaršek et al., 2012).
Removal of nitrogen compounds
We found that the pilot rain garden for storm runoff reaches its highest efficiency in total nitrogen and TKN removal when the runoff rates are lower than 0.91 L/min/m2, while the nitrate removal turned out to be less efficient even with runoff volume values lower than the denoted ones above. These values indicate that the system had a high rate of nitrogen mineralization that could be transformed into nitrate (NO3 -), which escaped the system by washing the upper layer of the soil, due to the low ionic interchange capacity, hindering the degree of retention of the soil, conversely to what happens with other nitrogenous compounds such as ammonia (NH+ 4) (Yang et al., 2012).
Considering all the above, we propose an empirical model (Figure 4) for the behavior of the nitrogen added to the rain garden, which is fixated as organic nitrogen and then oxidized from ammonium to nitrate, passing through a nitrite transitional state that is readily absorbed by the plants; however, the nitrate reduction could generate N2(g) transported to the atmosphere. Most of these processes are remediated by nitrogen-fixing microorganisms in the soil, which play a crucial role within the nitrogen cycle (Rascio and La Rocca, 2013). The analysis of the model´s results indicates that the rain garden removed 31.5 % of the nitrogen that entered the system, including a reduction in nitrite (6.5 %) and nitrate (6 %). For the TKN or organic nitrogen, the removal percent was 31.7 %.
Rain gardens are imperative for nitrogen fixation, which is an important process to avoid groundwater contamination and the eutrophication of superficial aquatic ecosystems (Chahal et al., 2016). In comparison with other systems proposed for temperate zones for nitrogen removal (Table 2), results indicate that our rain garden for tropical cities can gain higher values of nitrogen removal from runoffs. In addition, these results indicate that this process is variable, depending on several factors such as grain size distribution and geochemical features of the soil, as well as the composition and density of the plants used for the system (Fletcher et al., 2007; Hatt et al., 2009). For this reason, we suggest, in future studies, to make some modifications in substrate compositions and use additional species with similar features to the one used here.
Conclusions
The proposed rain garden represents an environmentally sustainable alternative for the management of residual water from tropical zones. It reduces the nitrogen concentration present, preventing almost all nutrients from entering aquatic ecosystems and the manifestation of eutrophication. Also, this model of garden facilitates the growth and maintenance of native plants in urban areas, improving the green areas that not just beautify the landscape but help to fix pollutants of global impact as CO2. For all these reasons, it is suggested to the scientific and academic community to continue researching and generating technological developments that maximize the efficiency of rain gardens in tropical zones, and to the governmental entities in charge of managing the cities located in these zones to contemplate the possibility to use this type of technology for the management of runoff water as an environmentally sustainable alternative.