Introduction
Tissue engineering, with applications in disease modelling and biomedical areas, remains the focus of scientific research (1), (2). Various scaffolds have been fabricated for these applications and have been studied from biological and mechanical perspectives (3). One of the most common base materials for tissue engineering is polycaprolactone (PCL) (4)–(6). Various PCL structures and configurations have been fabricated as scaffolds and used in vitro and in vivo for tendon, ligament, and skeletal muscle applications (4), (5), (7). These studies take advantage of the polymer’s biocompatibility and its elastic properties (4).
The most common fabrication techniques for PCL scaffolds include variations of additive manufacturing and electrospinning (5), (8)–(10). PCL scaffolds can be designed with different architectures and can be 3D printed in various configurations such as mats, meshes or porous structures (11)–(14). Baji et al (7) reviewed several experiments that focused on the fabrication of PCL filaments by electrospinning, ranging from 0.1 µm to 1.4 µm in diameter, and Ghobeira et al. (15) reported PCL scaffolds fabricated by a modified fused deposition modeling (FDM) method with random or aligned fiber configurations. Li et al (16) provided a review of the mechanics of single electrospun fibers, and Shanmugam et al. present a review for 3D printed fiber composites. Electrospinning is mainly used for nerval, ocular and bone tissue engineering (17). Other fabrication techniques rely on the thermoplastic properties of PCL, such as extrusion and melting-drawing techniques. These techniques are used to produce individual polymeric fibers. An et al (18) used a drawing technique to fabricate a bundle of PCL fibers that ranged from 10 µm to 25 µm in diameter and Visco et al. (19) fabricated PCL suture threads by extrusion with a resulting average diameter of 300 µm.
Skeletal muscular tissue, in nature, always reacts to force as an aggregate of fibers; therefore, its mechanical properties are a result of grouped fibers and they cannot be inferred from an individual muscular fiber (3), (20). When engineering a scaffold for skeletal muscle tissue engineering applications, it must mimic the skeletal-muscle physiology and its mechanical properties. The scaffold should aim to imitate the collective behavior of a muscle and have elastic properties. Moreover, in vitro cell growth occurs over time; therefore, the behavior of a scaffold under several hours of varying stress must be considered. Several authors such as Alexeev et al (4).;Baji et al. (7); Brennan et al.(21); and Kim et al (22) have described the monotonic mechanical properties of electrospun fibers; however, the cyclic properties of the fibers need to be studied further. Among the most common techniques for mechanical testing of microfilament samples is the use of grips that apply pressure on both ends of a specimen (23), cleat jigs or similar devices (24), (25), and using support material with an adhesive to fix the filament and attach the support to a measurement apparatus (26).
This work aims to investigate the monotonic and dynamic mechanical properties of a muscular tissue engineered scaffold fabricated with PCL using a grip system suited for this purpose. Extrusion was used to produce homogeneous PCL microfilaments to be used as samples. The filament was organized into bundles that mimic skeletal muscle using a tailor-made grip system. Monotonic tensile tests and stress-control cyclic tests were conducted on the fabricated PCL microfilaments to investigate the strain under different load controlled conditions. The results were used to understand and improve the fabrication, number, and behavior of polycaprolactone microfilaments to be used as bundles to model skeletal muscle. Various tests were conducted, and the mechanical properties of the microfilament bundles were estimated. The obtained information was used to understand, improve, and predict the behavior of PCL filaments to be used as a skeletal muscle model scaffold.
Materials and methods
Microfilament fabrication and surface characterizationMicrofilament fabrication and surface characterization
Polycaprolactone, PCL (Sigma-Aldrich, Mn 80000 g/mol) pellets were used to fabricate PCL microfilaments. A spool of filament was fabricated using an extruder (Filabot Ex2) at 80 °C with a 1 mm diameter die (see Figure 1 (a)) and collected with a spooler, (see Figure 1 (b)). Then, the filament was stretched until plastic yield, ε ∼97%. Five samples were chosen for monotonic tensile testing and six samples were selected for dynamic tensile testing.
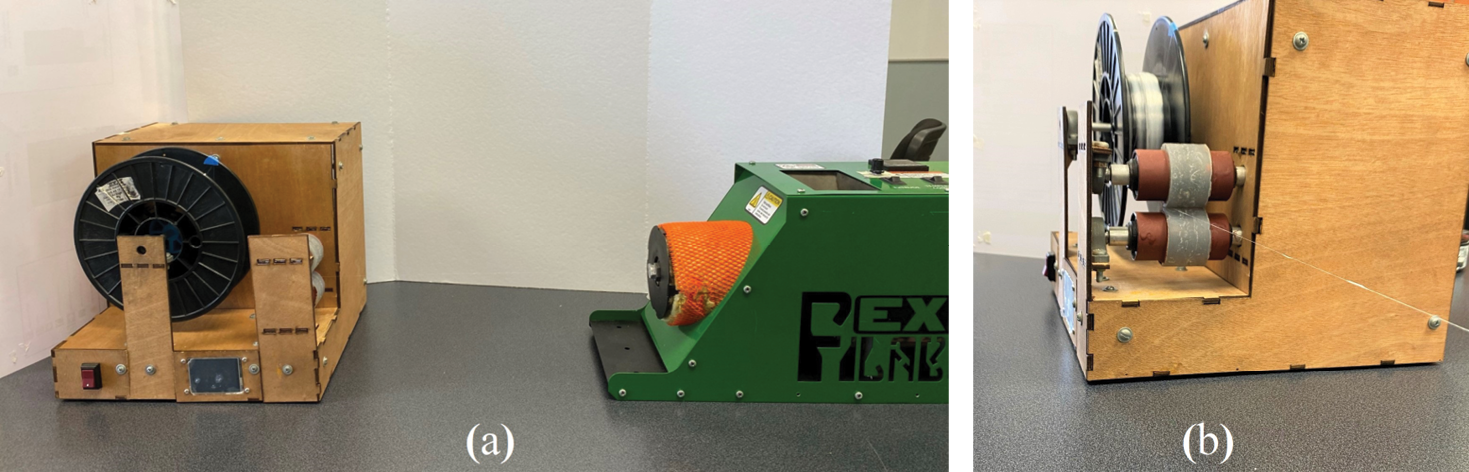
Figure 1. Fabrication technique of PCL filaments (a) Extruder and spool system (b) Spooler with filament.
Figure 1. Fabrication technique of PCL filaments (a) Extruder and spool system (b) Spooler with filament.
Mechanical testing
Monotonic tensile test
The mechanical properties of the microfilaments were evaluated with a testing and alignment adapter (figure 2) fabricated from 316 L stainless steel and designed to be attached to a load cell. Figure 2 (a) depicts the adapter components and figure 2 (b) shows the microfilament bundle held in the adapter. The assembly of the microfilaments on the testing adapter began by fixing them between the metal retainers; then, two turns were made around the first knob. Next, the microfilaments were carefully tied around both knobs, without adding extra tension. Then, the remaining end was tied twice onto the second knob and finally fixed between the second set of metal retainers.
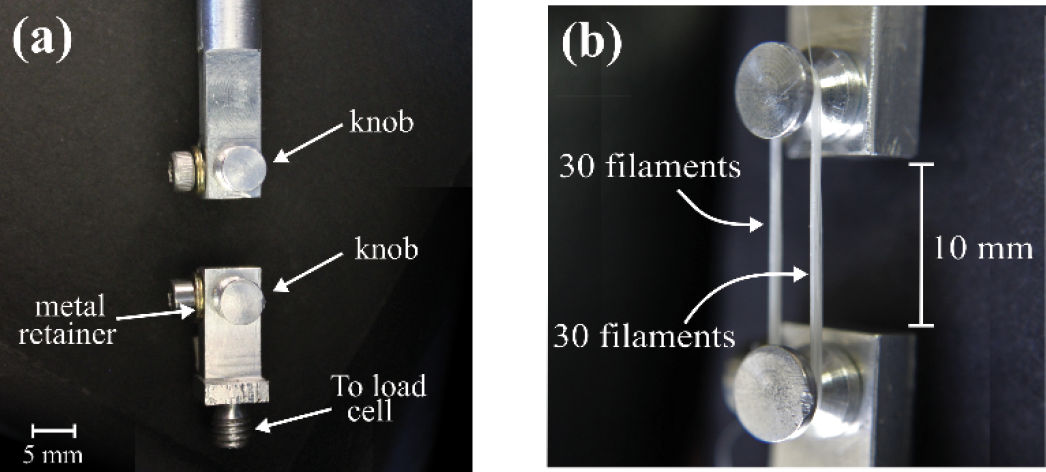
Figure 2. Tailor made grip adapter for mechanical testing of PCL microfilaments (a) Grip adapter with labels (b) Microfilaments tied to grip adapter.
Figure 2. Tailor made grip adapter for mechanical testing of PCL microfilaments (a) Grip adapter with labels (b) Microfilaments tied to grip adapter.
The testing adapter was placed on a servohydraulic test system (model 810, MTS System Corp) equipped with a 250 N load cell. Monotonic tensile tests were performed on five samples. The cross-head speed was set to 38 mm/min following the rate for suture materials according to the United States Pharmacopeia (USP) standards for tensile strength (27), and the test was carried out until rupture of at least one microfilament. The stress was calculated as the load divided by the equivalent area. The equivalent area corresponds to the sum of the cross-sectional area of the 60 individual filaments using the average diameter. The strain (ε) was the ratio between the height change (∆l = lf − l0) and the initial height (l0 =19 mm) between the knob’s centers.
The mechanical parameters of Young’s modulus (E), yield stress (σy), and ultimate yield stress (σult) were calculated. E was obtained as the slope of the linear section of the stress-strain curve, σy was obtained as the offset of 0.2% of the strain, and σult was calculated as the maximum value just before one of the filaments broke.
Dynamic tensile test
The PCL microfilament bundles were studied under a dynamic loading scheme. A test was designed to probe the deformation of the sample for 5.3 × 105 cycles (∼72 h). Cyclic tests were performed under load control with a sinusoidal signal at 2 Hz. The amplitude was selected from the elastic region of the microfilament behavior determined by the monotonic tensile test, and the maximum and minimum stress were calculated as stated previously. The selected amplitude corresponded to σmin= 52 MPa for all tests and σmax was set to σy, 0.9σy, 0.8σy, 0.7σy, or 0.6σy in each test. For each dynamic test, hysteresis loops were obtained, and several secant moduli (Esec) were calculated as the slope of the peak and valley values at selected cycles (see Table 1). Several cycles were selected to determine the progress of deformation throughout the test.
Table 1 Esec values and their corresponding cycle
Esec(i) | Cycle |
Esec1 | 10 |
Esec2 | 1500 |
Esec3 | 8100 |
Esec4 | 57100 |
Esec5 | 81500 |
Esec6 | 439300 |
Results and discussion
Microfilament fabrication and surface characterization
Figure 3a shows an individual, unfused fabricated microfilament, whose surface is mostly uniform and shows no fabrication defects. The microfilament can be arranged as parallel fibers or in woven configurations to produce different scaffolds for tissue engineering applications. This fabrication technique was versatile because different diameters can be fabricated by customizing the extruder’s die and spool speed.
Figure 3. Light micrography of a fabricated PCL microfilament at (100X)
An important advantage of the fabrication method implemented in this work is that no solvents were required, resulting in the chemical and superficial properties of the filaments remaining unchanged. This is important because the microfilaments maintain the biocompatibility and biodegradability of PCL (28). Kim et at and An et al have also fabricated PCL microfilaments without using any solvents with diameters that range from 9 to 25 µm. In contrast, other techniques require solvents that can be prejudicial for in-vitro applications. Solvent-based techniques can also be used to produce random fiber networks (5), (6).
Mechanical characterization of the microfilament’s bundles
Monotonic tensile test
A stress-strain curve of the microfilaments is shown in figure 5. The curve begins with a small toe followed by a linear elastic region. The change in slope is associated with plastic deformation of the microfilaments. After plastic deformation of approximately 22%, failure occurs by the rupture of one or more microfilaments; this is evident as a series of steep decreases in the stress values. Each curve represents the calculated stress for a group of 61 filaments placed in the grip system. Its mechanical parameters were calculated: the Young’s modulus was E =2184 ± 394 MPa, the tensile yield stress was σy =275 ± 31 MPa, and the tensile maximum stress was σult =316 ± 59 MPa. The measured elasticity of the microfilament group produced in this work is in the range of the elastic modulus of an animal tendon of ∼2000 MPa (29). Other authors have fabricated PCL microfilaments with diameters of ∼300 µm (19) and 6 µm(4), however, their monotonical mechanical properties were measured for individual threads or filaments; therefore, they cannot be compared directly with the microfilament bundles presented here, owing to differences in the measuring method and grip system. The tied configuration was selected because it results in a 3D structure that resembles the organization of a skeletal muscle.
Figure 5. Tensile stress-strain behavior for 5 bundles of PCL.
The elastic modulus of the microfilaments depends on their size, orientation, and distribution. The developed grip adapters were an important tool for mechanically testing the collective behavior of the microfilaments as independent, parallel, organized filaments with an adjustable initial gauge length. By adjusting the number of filaments or by modifying their diameter, the mechanical properties of the scaffold can be tuned. The grip adapter presents a reproducible and adequate technique that properly holds the sample and allows the mechanical properties of 3D scaffolds to be studied; also, it is a versatile design because tests can be conducted under tensile loads, torsional loads, or a combination of these. Additionally, several diameters of polymeric filaments, ranging from 10 µm to 2 mm, can be fixed using the adapter.
Dynamic tensile test
The dynamic response of microfilament bundles was studied under different loading conditions to determine the load range where filaments can be used for long term studies. Therefore, it was important to determine the mechanical conditions for which the filaments can be dynamically stressed. Figure 6(a) shows the mean strain σmean for PCL groups as a function of the cycle number (N), where each curve represents a different loading amplitude. The mean strain was calculated as σmean = |σmax + σmin|2−1. For σmean < σy, at the beginning of the test, N < 70 cycles, the microfilaments deformed quickly. Next, the strain increased around 2% during 10,000 cycles, and finally, when 100,000 cycles were reached, the filaments reached a steady state where strain remained roughly constant; for example, strain increased by only 1% from 100,000 to 530,000 cycles. Tissue engineering scaffolds with mechanical stimulation, such as bundled microfilaments, are required to retain their integrity for the duration of cellular reproduction and proliferation (22), (30). The results show that PCL microfilaments can be used under constant loading tests for at least 5.3×105 cycles, equivalent to 3 days. Therefore, the microfilament bundles are suitable for dynamic stimulation of cell-seeded microfilaments, as long as a controlled environment is provided. Figure 6(a) shows that filaments can be tested under a wide variety of loading schemes without losing their integrity, for dynamic loads less than its yield stress σmean < σy. The fabricated microfilaments can be studied under constant dynamic loading conditions or designed loading conditions that are either static or periodic under stress-controlled tests with a σmean value of 270 MPa.
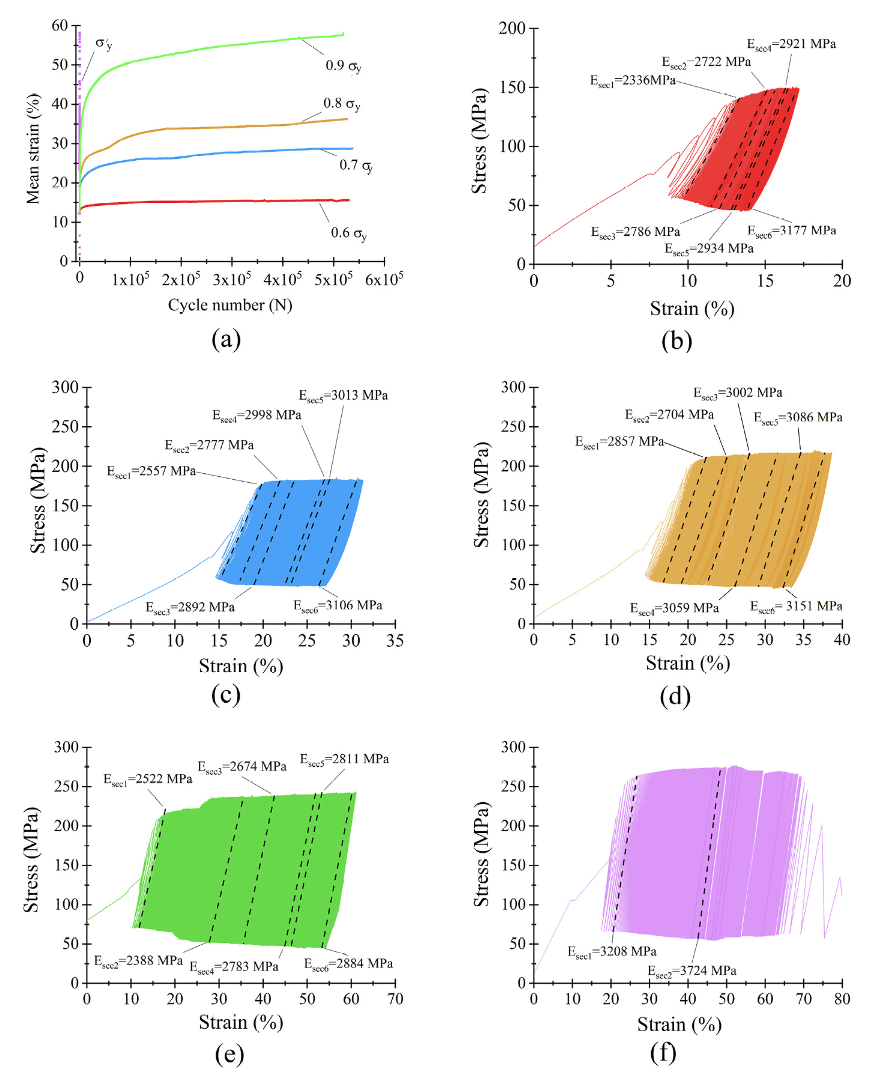
Figure 6 Dynamic response of microfilament groups under various loading scenarios (a) σmean as a function of N (b) σmax = 0.6σyc) σmax = 0.7σy (d) σmax = 0.8σy (e) σmax = 0.9σy (f) σmax = σy
Figure 6 Dynamic response of microfilament groups under various loading scenarios (a) σmean as a function of N (b) σmax = 0.6σyc) σmax = 0.7σy (d) σmax = 0.8σy (e) σmax = 0.9σy (f) σmax = σy
Figure 6 Dynamic response of microfilament groups under various loading scenarios (a) σmean as a function of N (b) σmax = 0.6σyc) σmax = 0.7σy (d) σmax = 0.8σy (e) σmax = 0.9σy (f) σmax = σy
The strain of the samples is directly related to the applied stress, as seen in figure 6(a). The highest strain reached was for 0.9 σy and the lowest was for 0.6 σy, as shown in table 2. For example, for σmax = 0.6 σy, the highest strain was 15% and a steady state was reached in less than 1.25×104 cycles. The difference between the end and the beginning of the steady state was less than 1%. When σmax = σy, samples lasted only 6,000 cycles.
The microfilaments exhibited permanent plastic deformation at the end of the test. Figures 6 (b),(f) show the hysteresis curve for the microfilaments for all σmax values. For σmax < 0.9σy no cyclic softening or hardening of the microfilaments wass present; this was evident because the amplitude remained between σmin and σmax. For σmax = 0.9σy, a small cyclic hardening occured when ε = 20% until the end of the test. For σmax = σy, there was a slight cyclic softening when ε = 47%. All PCL microfilament groups exhibited cyclic deformation accumulation; this was visible as the hysteresis curves shifted along the strain axis. The deformation accumulation was directly related to the applied strain, being the highest for σmax = 0.9σy.
Table 2. Maximum strain, steady state and plateau deformation for the loading conditions.
Maximum strain (%) | Steady state Cycles | Plateau deformation from 100,000 to 530,000 cycles (%) | |
σmax = 0.6σy | 15 | < 1.25×104 | 1 |
σmax = 0.7σy | 26 | 5×104 | 4 |
σmax = 0.8σy | 35 | 1.5×105 | 4 |
σmax = 0.9σy | 55 | 1.5×105 | 7 |
The secant moduli, for each stress-strain curve, are shown in table 3. Statistically, Esec1 to Esec6 had a normal distribution and equal variance (α = 0.05; p = 0.7). The percentage difference Esec% was calculated as Esec% = ((Esec6 − Esec1)·(Esec1)−1)·100 to monitor the stiffness evolution from the beginning to the end of the test. From σmax = 0.6σy to σmax = 0.9σy, Esec% was less than 2% of Esec(i); therefore, differences were small, suggesting that for each σmax, the stiffness of the microfilaments was relatively constant during the 5.3×105 cycles. The low amount of change in deformation, reflected by the small change in the secant modulus, indicates that the PCL microfilaments hold their mechanical elasticity and properties for at least 5.3×105 cycles. These characteristics indicated that the microfilaments were suitable for in vitro mechanical stimulation tests with different loading schemes. For instance, cell-loaded microfilaments can be subjected to different stress scenarios to study the resulting growth and proliferation of cells depending on the applied stress(31), (32).
Table 3. Calculated secant moduli of PCL microfilament samples at different times during fatigue testing.
Esec1 | Esec2 | Esec3 | Esec4 | Esec5 | Esec6 | Esec% | |
σmax = 0.6σy | 2336 | 2722 | 2786 | 2921 | 2934 | 3177 | 36 |
σmax = 0.7σy | 2557 | 2777 | 2892 | 2998 | 3013 | 3106 | 21 |
σmax = 0.8σy | 2857 | 2704 | 3002 | 3059 | 3086 | 3151 | 10 |
σmax = 0.9σy | 2522 | 2388 | 2674 | 2783 | 2811 | 2884 | 14 |
σmax = σy | 3208 | 3724 | Sample failed |
Conclusions
This study represents an initial phase towards achieving an in vitro, biomimetic, polymeric scaffold suitable for mechanical stimulation in a bioreactor. A simple technique was used to fabricate PCL microfilaments 90 µm in diameter that were uniform and had a smooth surface. Due to the fabrication technique the microfilament kept its chemical and superficial properties. A grip system was designed and tested to organize the microfilaments as bundles for mechanical testing. The evaluation of the static mechanical properties revealed an elasticity of 2184 MPa, similar to that of muscular tissue, and dynamic testing showed that the microfilaments endured stress-controlled fatigue for 5.3×105 cycles, while the stiffness of the filaments remained similar for the duration of the test. The fabricated microfilaments show promising mechanical properties for use as parallel aligned scaffolds for an in vitro mechanical stimulation assay in which several stress values could be adjusted for the required application. Moreover, the number of filaments in a bundle can be controlled depending on the application. The results of this study suggest that the PCL microfilaments may be promising for cell-growth and proliferation studies under mechanical loading scenarios.