Introduction
Current reef degradation is mostly a consequence of human-related activities. Rising sea temperatures and land-produced pollution and sedimentation threaten reefs capacity to provide invaluable ecosystem services to coastal communities (Matthews & Wynes, 2022). Scleractinian corals, mainly reef-building species, and by extension reefs, are in a ticking race against time, as recent models suggest irreversible damage if serious committed actions to achieve 1.5 °C goal are not taken before the year 2050 (Kleypas et al., 2021). While we as a society develop the strategies and the technology to mitigate the root cause (carbon emissions), it is paramount to buy time, by fostering reef recovery and corals'adaptive capacity (Baums et al., 2019).
Coral restoration is a novel management tool, that combines theories and concepts of marine ecology with terrestrial reforestation methods. In practice, restoration takes the form of coral gardening, a science-based two-step process consisting of (1) cultivating foundational coral species and (2) transplanting them in to denude parts of the reef (Rinkevich, 1995). The former can be done in site or in situ (typically involving some form of underwater nursery) or out of it -a.k.a. ex situ coral aquaculture-. Ex situ coral culture has proved to be a valuable restoration tool as it enables the mass production of coral fragments and larvae. For example, Shafir et al. (2001) carried out fundamental work, evaluating the feasibility of using 821 ''nubbins” or buds (tiny fragments of no more than 15 polyps), as an effective method in the production of propagative coral material. Pillay et al. (2012) compared the growth rates and survival of Pocillopora damicornis (Linnaeus, 1758) in an in situ and an ex situ nursery for two years, resulting in a significantly higher survival at the land base nursery. Although most propagation studies focus on fast-growing branching species, Forsman et al. (2006) investigated the effect of initial cutting size on the cultivation of massive coral fragments (Porites compressa Dana, 1846 and Porites lobata Dana, 1846), and found a significant positive relation between fragment size and growth, but no evidence of size-specific mortality. In 2015, Forsman and colleagues, expand this notion, characterizing not only coral fission properties but also exploring coral fusion restoration potential.
Parque Marino del Pacífico (PMP) is a department of the Ministry of Environment and Energy of Costa Rica (MINAE), design to bolster marine social-focused endeavors for the sustainable development of the Pacific coast (https://parquemarino.org). These includes, among other activities, the promotion of projects and research in marine aquaculture with emphasis on the production of commercial fish species through the Laboratory of Biotechnology and Marine Aquaculture.
The objective of our study was to describe ex situ coral culture viability (in terms of survival and growth) of the two-main reef-building species of the Pacific coast of Costa Rica, a massive species, P. lobata, and a branching coral, P. damicornis. We also described a method to account for production yield and present coral culture recommendations.
Materials and methods
Collection site: Playa Blanca fringing reef, with depths between 2-14 m, limits to the east with Playa Matapalo and to the west with Punta Matapalito (Méndez-Venegas et al., 2021). Five healthy ~15 cm2 P. damicornis coral colonies and one ~12 cm2 Porites lobata colony were collected during April 2018 by Scuba diving at Playa Blanca (10°32'04.5''N, 85°45'47.6” W), Guanacaste. The colonies were transported in a cooler with seawater and aeration to the Marine Aquaculture Research and Marine Biotechnology facilities at the Parque Marino del Pacífico, Puntarenas. Once in the wet lab, colonies were acclimatized (28°C) for one week and then fragmented (i.e., micro fragments between 1.5-2.0 cm2). A total of 180 coral fragments where generated and fixed to ceramic plugs. The surface of the ceramic plugs surrounding coral tissue was carefully scraped two times per week with a nylon toothbrush in order to prevent algal growth or biofouling accumulation. The experimental trial lasted for 152 days.
Fragments and tanks set-up: Fragments were randomly placed in one of six table-like grid structures (60 x 60 cm2), each holding 15 fragments of P. lobata and 15 fragments of P. damicornis. Coral tables were distributed between two tanks, for a total of 90 fragments (45 per species) in each tank. The 10 m3 circular fiberglass tanks were placed on a concrete slab under a mesh cover with 80 % sunlight filtration. Each tank was connected to a semi-closed recirculation system with 5 % continuous flow of fresh seawater from a common reservoir. The recirculation flow rate was 60 L/min. The systems were composed of a mechanical filter model Astral pool Aster 99, a biological filter (plastic canisters with 60 L of filtering material from plastic biobarrels), a three-phase centrifugal pump 230 v, 0.5 HP, a UV filter brand UV STERILIZIER and a foam fractionator RK2, model RK10AC-PF. In addition, two aeration stones and one HOBO temperature sensors per tank were placed. It was assumed that the tanks had the same conditions and therefore one would be the back-up of the other in case of fragments collapsed. Photosynthetic active radiation or PAR was measured using a Sper Scientific luxmeter model L860152. To determine the nutrient content (ammonium, phosphates, nitrates, nitrites) per tank, water samples were taken weekly and then analyzed using a Continuous Flow Autoanalyzer (FIA), at the Chemical Oceanography Laboratory, CIMAR, University of Costa Rica. Alkalinity, calcium, and magnesium were measured using commercial Aquaforest®. Salinity was measured at convenience using a refractometer.
Growth and survival: Photographs were taken every 15 days from May 17th to October 17th 2018 with an Olympus (TG-5) waterproof camera. Survival and fragment growth (as a conservative estimate of change in live tissue area) (Forsman et al., 2006) were estimated from these photographs, using ImageJ 1.45 free software (Collins, 2007) by calibrating pixel value statistics using fixed ceramic plug area (7.02 cm2), grid length (1.6 cm) and ceramic plug (0.5 cm). In the case of P. lobata, the area calculated from top view photographs corresponds to the total live tissue covering the ceramic plug, while for P. damicornis the area corresponds to the basal area (BA) of tissue attached to the ceramic plugs. The length (the maximum distance between the distal branches) and width (the measure perpendicular to the length) of P. damicornis branches were also calculated from top-view photographs. The height of each P. damicornis fragment was calculated from lateral photographs. Out of these three metrics, the crown area (CA) (Baums et al., 2019) and ellipsoidal volume (Vol) (Salinas-Akhmadeeva, 2018) for each P. damicornis fragment were calculated.
Survival percentage was calculated by subtracting the number of dead coral fragments from the total sample. Growth rate (GR) was calculated for the surviving fragments, as the difference between current (afraC) and prior measurement (afragP) divided by the days passed between each other (tC-Tp). (GR (cm2 d-1) = (afragC - afragP) / (tC - tP)).
Porites lobata culture yield: culture yield (Y) for this species was calculated as the yield obtained proportional to the expected yield, which equals 7.07 cm2 of live-coral tissue covering the ceramic plug. In order to achieve this, the following equations modified from Schippers et al. (2012) were used:
Where Resp is the expected yield (the desired harvest expressed in cm2), Nfrag is the total initial number of fragments to be grown, and Pfrag is the average harvest productivity per fragment during the culture period; L is suggested as the lag factor considering the survival (100 - % of surviving fragments that did not reach the desired harvest size/100), and Ft is the fragment size at the end of the harvesting period of duration t.
Statistical analyses: Wilcoxon rank sum test was used to establish significant differences between variables (except salinity, which was not systematically measured) per tank. Survival among species was compared using the Kaplan-Meir method (Lee, 1992). Growth data was transformed to log10 and fit into general linear regression models. Spearman's correlation analysis was conducted to determine the relationship between crown area (CA) and volume (Vol). All statistical analyses were conducted at 95 % confidence level using Rstudio free software (Grömping, 2015).
Results
Survival: During the third week of October 2018 all fragments in tank 1 (T1) died due to freshwater entering the culture system as a consequence of extreme rainfall events (Morera-Rodríguez, 2018). Prior to this event, the overall survival for P. lobata fragments was 68.89 % in T1 compared to 71.11 % in tank 2 (T2). In the case of P. damicornis, 71.11 % of fragments survived in T1, while in tank 2 survival was 100 %. Regardless of the species, a significant difference was found in the total survival of the fragments placed in T1 with respect to those placed in T2.
Porites lobata growth: Average initial area of P. lobata was 1.31 ± 0.37 cm2 and 1.36 ± 0.31 cm2 for fragments placed in T1 and T2, respectively. After 152 days, fragments in T1 had doubled their area to a total of 2.83 ± 1.07 cm2 (216 % increase), at an average growth rate of 0.010 ± 0.052 cm2 d-1. It is worth noting that rapid coenosarc formation and tissue expansion (~ 1 cm2) occurred during the first month where fragments grew the fastest (0.069 ± 0.026 cm2 d-1) until reaching a plateau at day 44, from which both growth and growth rate decreased slightly but steadily until the end of the experimental essay (Fig. 1).
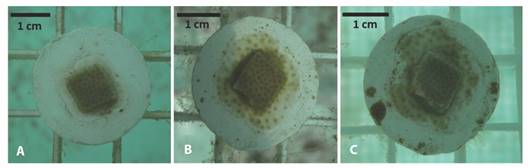
Fig. 1 An example of the growth of a Porites lobata fragment placed in T1; A. Expansion of the coenosarc (13 days); B. tissue with visible polyp formation (42 days); C. loss of tissue in the periphery of the fragment (55 days).
The fragments of P. lobata in T2 experienced a rapid increase in area from 1.36 ± 0.31 cm2 to 2.06 ± 0.69 cm2 at 0.039 ± 0.053 cm2 d-1 rate during the first month; reaching a maximum of 4.43 ± 1.55 cm2 at day 113, from which shortly after, growth started to decreased, resulting in a final area of 3.78 ± 1.55 cm2 (277 %) (Table 1). In addition, seven T2 fragments were able to fully cover the ceramic plug area in ~ 90 days.
Table 1 Change in total number, percent survival, and growth values associated with P. lobata during the experimental period.
Tank | Initial N | Final N | Survival* | Initial fragment area* (cm2) | Final fragment area*(cm2) | Average increased (%) | Growth rate* (cm2 d-1) |
---|---|---|---|---|---|---|---|
T1 | 45 | 31 | 68.89 % | 1.31 ± 0.37 | 2.83 ± 1.07 | 216 | 0.010 ± 0.052 |
T2 | 45 | 32 | 71.11 % | 1.36 ± 0.31 | 3.78 ± 1.55 | 277 | 0.016 ± 0.0.43 |
Statistical significance (p-value) | 5 x10-08 | 0.002 | 0.0008 |
* Symbol denotes significant differences (P-value < 0.05) between tanks.
Linear regression indicates that change in area through time, for P. lobata fragments varied significantly (p-value < 0.005) as a function of the tank in which they were placed, although the residence time in the tanks alone explained only 16 % of the variation in growth (r2 = 0.16), and 18 % of growth rate (r2 = 0.18) (Fig. 2).
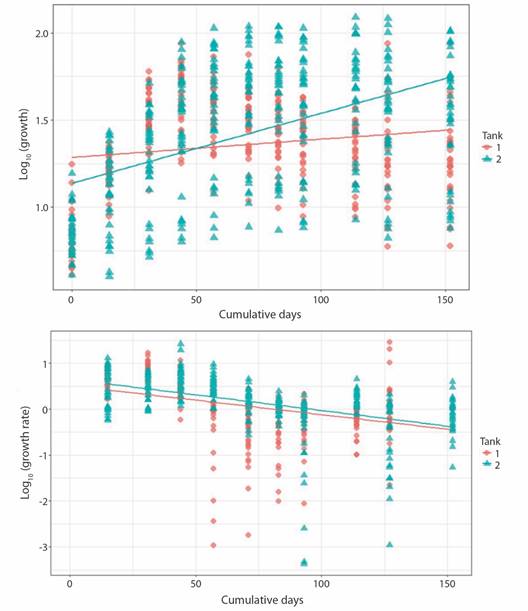
Fig. 2 Porites lobata fragments growth (upper panel, P-value < 2.2 x10-16, r2 = 0.16) and growth rate (lower panel, P-value< 2.2 x10-16, r2 = 0.18) related to days at tank 1 and tank 2.
Porites lobata culture yield: Yield (desired harvest of live tissue coral area) was calculated for T2 fragments that reached harvest size (7.07 cm2 or a fully cover ceramic plug). For an initial number of 45 Porites fragments (desired harvest) would be 318.15 cm2. Lag value (Z) was 0.78 (100-78 % of surviving fragments that didn't reach culture size during the experimental period/100). Average productivity per fragment was 1.55 (Pfrag = 1-0.78 x 7.07 cm2). Actual yield obtained was 69.75 cm2 (45 x 1.55); 21 % of total biomass that formerly enter the aquaculture system (Fig. 3).
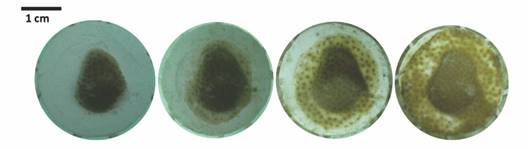
Fig. 3 An example of the growth of a P. lobata fragment placed in T2. From left to right, growth from May 17 to August 9 (82 days).
Pocillopora damicornis growth: Growth pattern varied between fragments. Some fragments'basal area managed to cover the majority of the ceramic plug, but had poor branch development; while the opposite was true for other fragments. Hence, the high standard deviation for growth metrics values. Basal area for T1 fragments increased from 0.57 ± 0.15 cm2 to 4.36 ± 2.01 cm2 at an initial growth rate of 0.032 ± 0.030 cm2 d-1 that notably decreased (0.001 ± 0.001 cm2d-1) towards the end of the experiment (Table 2). T2 fragments increased their BA from 0.59 ± 0.24 cm2 to 5.76 ± 1.29 cm2 at an initial growth rate of 0.026 ± 0.024 cm2 d-1 which then decreased to 0.005 ± 0.015 cm2d-1 (Fig. 4).
Table 2 Change in total number, percent survival, basal area (BA) and crown area (CA) values associated with P. damicornis during the experimental period.
Tank | Initial N | Final N | Survival* (%) | Initial BA (cm2) | Final BA (cm2) | Average BA increased (%) | Initial CA* (cm2) | Final CA* (cm2) | Average CA increased (%) | Initial Vol* (cm3) | Final Vol* (cm3) | Average Vol increased (%) |
T1 | 45 | 32 | 71.1 | 0.57 ± 0.15 | 5.59 ± 1.86 | 980 | 1.50 ± 0.76 | 5.49 ± 4.01 | 366 | 11.12 ± 6.8 | 58.61 ± 53.75 | 527 |
T2 | 45 | 45 | 100 | 0.59 ± 0.24 | 5.76 ± 1.30 | 976 | 1.46 ± 0.93 | 4.20 ± 3.12 | 287 | 8.69 ± 6.24 | 37.0 ± 37.86 | 426 |
Statistical significance (p-value) | - | - | < 2.2 x10-16 | 0.8176 | - | 3.08e-10 | - | < 2.2 x10-16 | - |
* Symbol denotes significant differences (P-value < 0.05) between tanks.
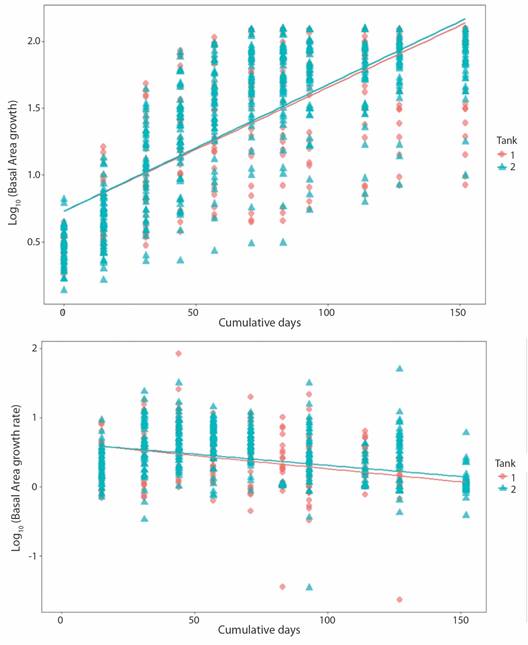
Fig. 4 Pocillopora damicornis basal area growth (upper panel; P-value = < 2.2 x10-16, r2 = 0.56) and growth rate (lower panel; P-value = < 2.2 x10-16, r2 = 0.14) related to days at tank 1 and tank 2.
Crown area increased from 1.50 ± 0.76 cm2 to 5.49 ± 4.01 cm2 at a rate of 0.023 ± 0.053 cm2 d-1 in the case of T1 fragments; change in CA for T2 fragments was from 0.59 ± 0.24 cm2 to 4.20 ± 3.12 cm2 at 0.019 ± 0.048 cm2 d-1. Growth rate for both sets of fragments decreased notably towards the end of the experiment. Final growth rate was 0.001 ± 0.010 cm2 d-1 and 0.015 ± 0.022 cm2 d-1 for T1 and T2, respectively (Fig. 5). Obtain values for volume behaved analogously to the CA values (Fig. 6).
Contrary to CA and Vol, no significance difference was found in BA values between tanks. Initial CA, Vol and growth rates values in T1 fragments were slightly higher than T2 fragments. However, growth rate in T1 decreased over time, as in T2 remained constant. As with Porites fragments linear regression indicates that tank factor alone is not a sufficiently robust predictive variable to explain difference between growth (T1/T2: r2 = 0.23) and growth rate (T1/T2: r2 = 0.006) among tanks. As expected, the same trend is observed for Vol (Fig. 4, Fig. 5, Fig. 6).
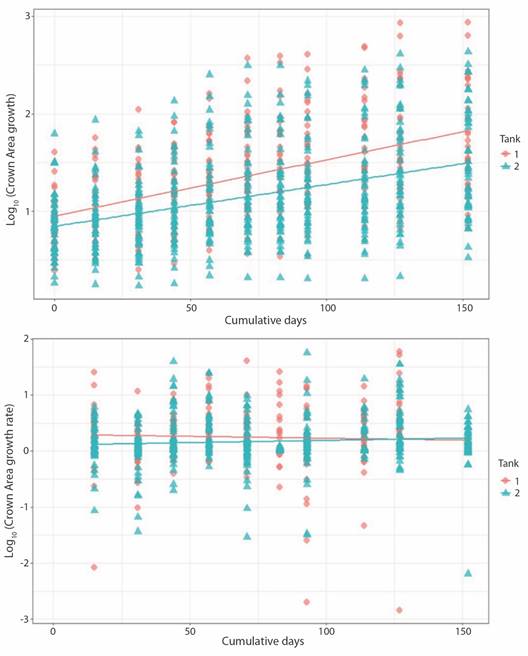
Fig. 5 Pocillopora damicornis crown area growth (upper panel; P-value = < 2.2 x10-16, r2 = 0.23) and growth rate (lower panel; P-value = 0.05582, r2 = 0.006) related to days at tank 1 and tank 2.
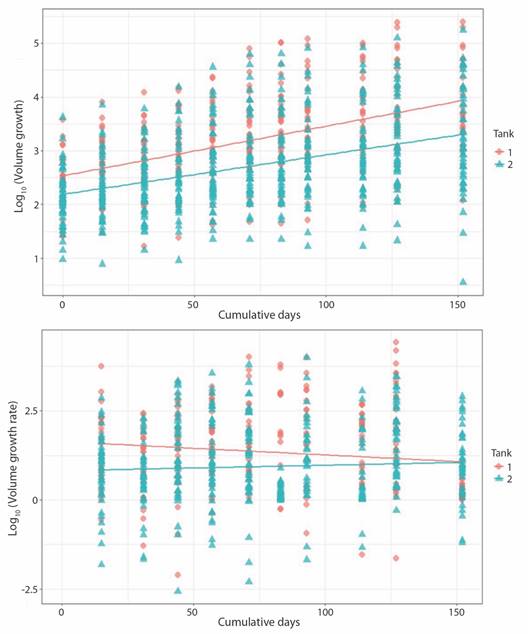
Fig. 6 Pocillopora damicornis volume growth (upper panel; P-value = < 2.2 x10-16, r2 = 0.26) and growth rate (lower panel; P-value = 6.001e-06, r2 = 0.03) related to days at tank 1 and tank 2.
The majority of the physicochemical variables showed significant differences between tanks (P-value = 0.005), except for alkalinity. A significant difference was obtained between temperature and PAR, both conservative properties. The majority of physicochemical parameters oscillated around the recommended lower limit, with the exception of temperature (Table 3, Table 4).
Table 3 Monthly value of physical-chemical parameters per tank.
Average values per tank (Tank 1/Tank 2) in a monthly basis | Temperature* (°C) | Alkalinity-KH (µmol/L) | Calcium (mg/L) | Magnesium* (mg/L) | PAR* (µ E.m-2.s-1) | ||||
Reference values (Borneman, 2008; Kleypas et al., 1999) | 26-30 | 8 | 425 | 1250 | 250-1000 | ||||
May | T1 | 29.42 ± 0.44 | 7.9 ± 0.14 | 392.5 ± 45.96 | 1175 ± 49.49 | 445 ± 132.23 | |||
T2 | 29.06 ± 0.72 | 7.2 ± 0.14 | 392.5 ± 60.10 | 1175 ± 49.50 | 476 ± 129.40 | ||||
June | T1 | 29.18 ± 0.48 | 7.6 ± 0.5 | 400 ± 0.5 | 1260 ± 0.5 | 1212 ± 34.41 | |||
T2 | 28.80 ± 0.47 | 6.2 ± 0.5 | 410 ± 0.5 | 1240 ± 0.5 | 1361 ± 138.35 | ||||
July | T1 | 29.26 ± 0.04 | 7.15 ± 0.21 | 392.5 ± 10.60 | 1255 ± 7.07 | 688 ± 514.77 | |||
T2 | 28.69 ± 0.44 | 7.4 ± 0.55 | 390 ± 14.14 | 1230 ± 0.5 | 699 ± 617.53 | ||||
August | T1 | 29.23 ± 0.13 | 7.0 ± 0.5 | 355 ± 7.07 | 1185 ± 21.21 | 1060 ± 251.49 | |||
T2 | 28.97 ± 0.003 | 6.63 ± 0.40 | 373.3 ± 23.09 | 1210 ± 14.14 | 610 ± 16.26 | ||||
September | T1 | 29.10 ± 0.30 | 6.2 ± 0.5 | 340 ± 0.5 | 1230 ± 0.5 | 1440 ± 468.29 | |||
T2 | 28.86 ± 0.26 | 6.7 ± 0.5 | 360 ± 0.5 | 1220 ± 0.5 | 671 ± 147.07 | ||||
October | T1 | 27.60 ± 0.26 | 4.56 ± 0.87 | 280 ± 0.5 | 860 ± 180.83 | 1171 ± 537 | |||
T2 | 27.41 ± 0.41 | 6.2 ± 0.5 | 340 ± 0.5 | 1110 ± 0.5 | 1267 ± 486.96 | ||||
Total (average values during experimental trial) | T1 | 29.09 ± 0.56 | 6.50 ±1.39 | 350.9 ± 51.80 | 1118 ± 188 | 895 ± 414.55 | |||
T2 | 28.74 ± 0.55 | 6.6 ± 0.87 | 368 ± 44.2 | 1156 ± 124.6 | 900 ± 410.31 | ||||
Statistical significance (p-value) | <2.2 x10-16 | 0.2133 | <2.2 x10-16 | 0.0192 | 0.001693 |
* Symbol denotes significant differences (P-value < 0.05) between tanks.
Table 4 Monthly value of nutrients per tank.
Average values per tank (Tank 1/Tank 2) in a monthly basis | Phosphates (µmol/L) | Nitrates (µmol/L) | Nitrites (µmol/L) | Ammonium (µmol/L) | |
May | T1 | 0.37 ± 0.22 | 1.90 ± 0.31 | 1.53 ± 0.44 | 7.70 ± 0.04 |
T2 | 0.36 ± 0.21 | 1.49 ± 0.38 | 1.25 ± 0.28 | 6.10 ± 6.61 | |
June | T1 | 0.40 ± 0.12 | 1.97 ± 0.15 | 1.59 ± 0.12 | 8.67 ± 4.5 |
T2 | 0.40 ± 0.09 | 1.81 ± 0.41 | 1.48 ± 0.33 | 11.20 ± 5.1 | |
July | T1 | 0.33 ± 0.01 | 2.19 ± 0.39 | 3.68 ± 2.71 | 5.80 ± 1.78 |
T2 | 0.32 ± 0.06 | 1.65 ± | 5.85 ± 6.38 | 6.99 ± 2.57 | |
August | T1 | 0.31 ± 0.01 | nd | 9.73 ± 1.59 | 6.36 ± 0.73 |
T2 | 0.37 ± 0.07 | nd | 8.17 ± 2.56 | 5.12 ± 0.94 | |
September | T1 | 0.37 ± 0.05 | nd | 8.96 ± 1.24 | 5.61 ± 2.19 |
T2 | 0.36 ± 0.05 | nd | 9.23 ± 1.46 | 5.20 ± 1.89 | |
October | T1 | nd | nd | 7.85 ± | 3.142 ± |
T2 | nd | nd | 4.98 ± | 6.77 ± | |
Total | T1 | 0.35 ± 0.05 | 2.0 ± 0.15 | 5.19 ± 3.6 | 6.34 ± 2.87 |
T2 | 0.39 ± 0.08 | 1.61 ± 0.38 | 5.34 ± 4.15 | 7.08 ± 4.23 | |
Statistical significance (p-value) | 0.8439 | < 2.2 x10-16 | 3.378 x10-11 | 0.0001413 |
nd denotes not detectable.
Discussion
Porites lobata survival rates for both tanks were consistent with those reported in Forsman et al. (2006) which range between 78-92 %. In the case of P. damicornis, Pillay et al. (2012) reported survival rates of 100 %; comparable to the survival of fragments in T2 in this study.
Fragment survival varied between coral species and culture tank. Fragment death occurred during the first two weeks of culture, regardless of the species. This is not surprising since the period subsequent to subclonation of donor colonies is one of most sensitive for the survival of small fragments, susceptible to predation, sedimentation and/or disease (Forrester et al., 2013; Lizcano-Sandoval et al., 2018; Rinkevich, 2005; Shafir et al., 2001). The latter was especially true for P. damicornis in T1, which presumably suffered from rapid tissue necrosis, a common disease in the realm of coral husbandry, and for which there is no known trigger; although it is typically associated with stress (Calfo, 2001; Luna et al., 2007).
The intraspecific variability in P. damicornis survival could be due to intrinsic factors, such as genetics differences, physiological state, previous health status, and even the area of the colony from which the fragments were extracted (Kenkel & Matz, 2016; Tagliafico et al., 2018; Yap, 2004). In his coral husbandry compilation manual, Bartlett (2003) warns that there can be significant differences between species within the same genus, and even between colonies of the same species.
For ex situ reared corals, mortality is usually the result of incidental herbivory by unwanted commensals, mainly nudibranch gastropods and Drupella snails (Gochfeld & Aeby, 1997; Forsman et al., 2006). No commensal organisms were observed during the 152 days of the experimental trial, corroborating the effectiveness of the filter system. Another reason related to potential mortality is the direct effect of nutrients on the algal growth; Yap & Molina (2003) report survival rates for Porites as low as > 20 %, attributed to competition derived from excessive growth of macroalgae in culture tanks with high nutrient levels. Although proliferation of macroalgae inside the tanks was notorious, the frequent cleaning of the ceramic plugs avoided possible fragment demise derived from excessive algal overgrowth.
Coral bleaching and death of Stylophora pistillata (Esper, 1792) fragments has been recorded after exposure to salinity levels of 15-18 PSU for 24h (Kerswell & Jones, 2003). Corals in T1 bleached and die over the course of 48 hours, presumably as the result of osmotic shock (Hoegh-Guldberg, 1999), due to heavy rainfall exposure which caused salinity to drop to 17 PSU.
Regarding growth, P. lobata fragments increased their area 216-277 % in 152 days; concurring with previous studies that report increases in area up to 228 % in 119 days and 357 % in 205 days (Forsman et al., 2006; Forsman et al., 2015). While it is true that the increase in area in this study and that reported by Forsman et al. (2006) are similar, the path that led to them was highly contrasting. In Forsman's results growth remains steady, whereas in this case, fragments grew exceedingly fast after stimulation by cutting during the fragmentation process (Page et al., 2018), yet after 44 days in the tanks the newly-form tissue began to slough off in most of the fragments, reflecting and overall decrease in growth and negative growth rate values. Thus, it is conceivable that the larger area recorded for fragments in T2 did not necessarily mean that they grew faster than those in T1, but that they deteriorated less as they were able to (1) maintain tissue integrity longer and/or (2) repair damage at the rate at which it accumulated (Kirkwood, 1981). It could be hypothesized that, while the biological filters and protein skimmer kept the water free of undesirable biological hazards, they may have simultaneously limited the supply of organic nutrients (i.e. zooplankton) and dissolved organic carbon in the tanks, which could have affected coral performance. Therefore, the inability of some fragments to sustain their newly-produced tissue could have been due to the lack of supplementary nutrition, forcing corals to catabolize their energy reserves, first lipids and in an extreme case, tissue (Gates & Edmunds, 1999; Kirkwood, 1981). This may have been the case for P. lobata in this study, since under stress conditions this species does not reduce metabolic demand, but rather relies on its abundant lipid reserve (Levas et al., 2013); a reserve that could not be replenished in a culture medium lacking a heterotrophic energy source.
When compared with wild colonies growth rate, ex situ reared fragments grew 12.2 mm year-1 (√ (0.013 cm2 per day x 365 days a year / π) x 10 mm; according to Forsman et al., (2006)) in contrast with 15.3-19.3 mm year-1 (Guzmán & Cortés, 1989). Growth in the culture tanks was comparable to growth in the field, but was far from profitable in terms of aquaculture, which is the premise upon investment in ex situ culture is based. Only seven Porites fragments fully covered the ceramic plug, reaching culture size at a rate of 25.05 mm year-1. This means a culture yield of just 21 % (69-75 cm2) of the desired coral harvest (318.15 cm2). In a hypothetical restoration/production scenario, this result would imply mayor investment losses, as one would expect a minimum yield of 200 % in order to sustain production, as part of the harvest is used to replenish the next production cycle (Leal et al., 2016; Osinga et al., 2011; Schippers et al., 2012).
The speed at which corals can attach to the substrate is often the first bottleneck in in situ coral restoration projects (Forrester et al., 2011; Guest et al., 2011; Tagliafico et al., 2018). Attachment rate varies according to region, environmental conditions, methodology and species (Clark & Edwards, 1995). For example, after one year of monitoring on the island of Bali, Endo et al. (2013) reports an attachment of 53 % for colonies of P. damicornis, in contrast, Guzman (1991) observed the formation of attachment points in less than 5 months for Pocillopora, placed on iron rods on Caño Island. No significant differences were found between tanks for attachment rate of Basal area. On average, it took the fragments 70 days to cover 63 % of the ceramic plug, which compares to Guest et al. (2011) findings, whom reported tissue extension over 66 % of the substrate in 82.5 days.
The gradual decrease in the growth rate of Basal area responds to the fact that once fixed and stable on the substrate, Pocillopora coral colonies reorganize its shape until it reaches the compact bush configuration that characterizes it (Guest et al., 2011; Rinkevich, 2000).
Correlation results suggest both crown area and volume share the same degree of association with the independent variable ''accumulated days”. Since the majority of scientific literature uses length and area, over volume as dimensional metrics for coral growth, Pocillopora growth results are discussed in terms of crown area (cm2). Needless to say, the use of one metric over the other will depend on the research's question. For example, studies focusing on biota-assemblage associated with coral colonies (Doszpot et al., 2019; Salinas-Akhmadeeva, 2018) will benefit from using volumetric measurements over area. The difference between T1 and T2 fragments initial and final growth rate, indicates growth rate was affected by the culture medium. T2 fragments growth rate remained relatively constant, in accordance with Kinzie & Sarmiento (1986) hypothesis which states: P. damicornis growth rate is independent of colony size, remaining constant over time.
In contrast to these argument, Lizcano-Sandoval et al. (2018) report that growth rate is determined by fragment size, the larger the size, the greater the growth, however, these authors compare growth rate between different size groups, rather than the change in growth rate of fragments of the same group size as they grew. Either way, studies focused on P. damicornis growth kinetics will hopefully settle this question.
Pocillopora genus is characterized by encompassing fast-growing species (Muko & Iwasa, 2011; Schlöder & D'Croz, 2004). On Caño Island an annual growth of 29.8 mm yr-1 was reported, similar to that one in Panama (27.8 mm yr-1) and Mexico (21.9 mm yr-1) (Gómez, 2014; Manzello, 2010). Whilst growth rates of 50 mm yr-1 were reported for Bahía Culebra, the highest in the Eastern Tropical Pacific (Jiménez & Cortés, 2003). In the Mexican Pacific, Tortolero-Langarica et al. (2019) and Tortolero-Langarica et al. (2020) report growth rates for P. damicornis fragments transplanted directly on the reef of 23.1 and 44.7 mm yr-1. When convert to length, estimate growth rate (~ 15.5 mm yr-1; as a result of Forsman formula) in this study is low compared with colonies growing in the wild, but similar to the 13.1 ± 15 mm yr-1 reported for P. damicornis growing in an open water flow culture system (Pillay et al., 2012).
Given the multiplicity of factors having a potential effect on coral fragments performance, isolating and determining the individual or combined effect(s) of any of these variables on both species is beyond the scope and objectives of this study. Nevertheless, the differences observed between fragments performance among tanks, can and should be cautiously explored.
Both tanks were adjacent to each other and received water from the same marine reservoir. Therefore, the contrasting results between one tank and the other may have been due to significant differences in conservative properties, such as temperature, PAR and precipitation, all influenced by shading and tank exposure. Another hint towards this hypothesis is that P. damicornis in T1 (more exposed), developed on average a bigger crown area compared with T2 fragments (less exposed). In the long run, small ''overlooked” differences in these physical variables would have had an effect in non-conservative factors (e.g., ammonium values change with biological activity) (Li et al., 2017; Silva et al., 2009), causing noticeable differences between sea water properties in each tank, which would have had an effect on coral fragments.
Nutrient and alkalinity (Kh) values were found to be on the low end of recommended range (Bartlett, 2013), and may have had negatively influenced coral growth by disturbing coral-zooxanthellae dynamics (Grover et al., 2003) or/and via macro algae proliferation enhancement.
This study describes base-line findings for scleractinian coral culture at Parque Marino del Pacífico aquaculture module. Both survival and fragment growth varied between species (P. lobata and P. damicornis) and culture tank, only few fragments show significant stable growth. Hence, culture tanks current set up and water conditions provided a suboptimal medium for effective coral rearing, there is a need for more studies regarding the relationship between species-specific growth kinetics, optimal culture conditions and cost-effective production. These results do not discredit coral aquaculture efforts. On the contrary, we hope they demonstrate the potential for refining ex situ coral culture practice in Costa Rica, and to be a starting point, from which to optimize culture conditions and methods into a viable tool for the country's reef systems effective management.
Ethical statement: the authors declare that they all agree with this publication and made significant contributions; that there is no conflict of interest of any kind; and that we followed all pertinent ethical and legal procedures and requirements. All financial sources are fully and clearly stated in the acknowledgements section. A signed document has been filed in the journal archives.