Mangroves are coastal ecosystems in the transition areas between marine and terrestrial environments, of well-known ecological, economic and social importance (Madi, Boeger, & Reissmann, 2015). Mangroves occur in tropical and subtropical regions. They constitute authentic coastal forests, in which plant structure and ecology are highly adapted to topographic and geomorphological differences, saline fluctuations and tidal amplitudes (Santos et al., 2012). Mangroves forests may cover estuarine areas, bays, inlets, river mouths, lagoons, or simply be exposed to the coastline (Schaeffer-Novelli, 1995).
The diversity of mangrove tree species is extremely low when compared to other tropical ecosystems. It comprises ca. 54 plant species considering mangroves worldwide distribution (Tomlinson, 1986). In Brazil, seven tree species are present, and belong to the Rhizophoraceae, Acanthaceae and Combretaceae families (Schaeffer-Novelli, Cintron-Molero, Adaime, & Camargo, 1990). Specific to its Southernmost distribution, only three tree species compose mangroves forest in Southern Brazil: Avicennia schaueriana Stapf & Leachman (Acanthaceae), Laguncularia racemosa (L.) Gaertn (Combretaceae) and Rhizophora mangle L. (Rhizophoraceae) (Schaeffer-Novelli et al., 1990; Kilca, Alberti, Souza, & Wolff, 2011; Soares, Tognella, Cuevas, & Medina, 2015).
Along the Brazilian coast, mangrove forests have different structure and species distribution (Lima & Tognella, 2012). For example, although L. racemosa distribution ranges over the coast, its representativeness is greater in Southern mangroves (Schaeffer-Noveli et al., 1990). Usually, L. racemosa can be found in open areas, as well as in transitional areas of restinga and lowland forests (Bernini & Rezende, 2003). The transitional forests are less affected by tides regime and are subject to less frequent flooding located inland (EMBRAPA, 2013).
Despite of its high primary production (Ball, 1996), mangroves are stressful environments to plant development due to high water salinity influenced by periodic tidal flooding, low oxygenation, and unconstrained soil (Lüttge, 2008). The vegetation associated to this ecosystem has specific adaptations to establish and thrive in halophytic environments (Krauss, Lovelock, McKee, López-Hoffman, Ewe, & Sousa, 2008), resulting in a set of adaptations in their structural, ecophysiological and functional characteristics (Pascoalini, Falqueto, & Tognella, 2014; Larcher, Boeger, & Sternberg, 2016).
Mangrove tree species may present three different mechanisms to cope with high salinity and to control salt concentration in their tissues (Parida & Jah, 2010). L. racemosa shows an ecophysiological mechanism of salt exclusion, which may be associated with a wide range of tolerance to salinity: L. racemosa occurs at contrasting salinities, in areas of salinity close to fresh water towards salt water (Sobrado, 2005). This mechanism is also reflected in its morphological and anatomical adaptations. For example, Bartz, Melo Jr., and Larcher (2015) suggests mangrove individuals are smaller, with lower trunk diameter height and crown density and higher leaf area, than individuals of transitional forests.
The high evaporative demand combined with the vulnerability to embolism or cavitation, due to the high concentration of ions in the environment, were previous associated to adaptations in the secondary xylem of mangrove species (Lovelock et al., 2007; Krauss et al., 2008). At high salinity, the low soil water potential requires a decrease in the plant water potential to maintain water uptake by roots, leading plants to decrease water conductivity in secondary xylem (Reef & Lovelock, 2015). The anatomical characteristics and, consequently, the physical-mechanical properties of this tissue, is affected by environmental variations (Baas, Wheeler, & Fahn, 1983; Carlquist, 2001; Marcati, Angyalossy-Alfonso, & Benetati, 2001; Cosmo, Kuniyoshi, & Botosso, 2010).
We expected mangroves species, as L. racemosa, to express several secondary xylem phenotypes in response to variation of environmental factors, such as precipitation, temperature and water availability. Thus, this study aimed to evaluate the wood anatomy of L. racemosa populations occurring in mangrove and transitional forests between mangrove and restinga forest. We hypothesize that mangrove individuals may have anatomical adaptations to deal with higher pore water salinity and lower soil water potential, while transition mangroves are less conservative in water use.
Material and methods
Study area: The Caieira Municipal Natural Park was located in the center-east portion of the city of Joinville/SC, Brazil (Figure 1). Inside the park, there was the Lagoa of Saguaçu (26°18’24’’ S - 48°47’36’’ W), with approximately 1 000 km² of mangrove remnants and transitional forest, from mangrove towards restinga and lowland forest. The transitional forest occured between the mangrove zone and restinga forest; is not directly subject to the tides regime (not frequently flooded), and is characterized by the presence of the species Talipariti tiliaceum (L.) Fryxell (Malvaceae) as a schrub (EMBRAPA, 2013). L. racemosa had high absolute density in both mangrove and transitional forests.
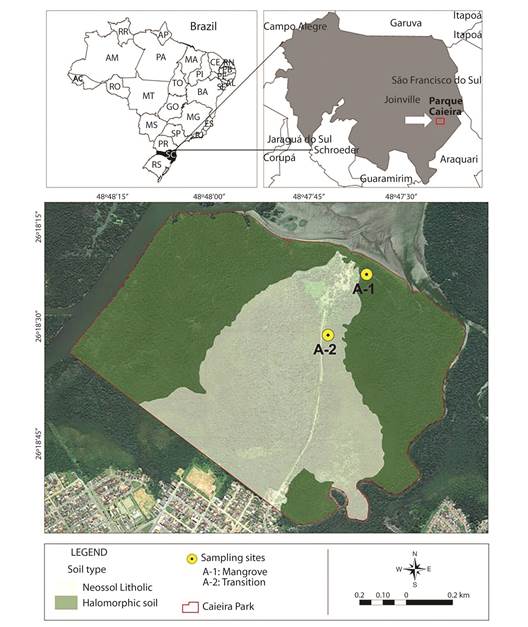
Figure 1 Study area inside the Caieira Municipal Natural Park, Joinville, Santa Catarina, Brazil: Mangrove forest (A-1) and transitional forest (A-2) (located between lat -26o 18’ 15”/-26o 18’ 30” and long -48o 48’/-48o 47’ 30”).
The holomorphic soils of mangrove forests were developed by the deposition of marine and fluvial sediments, with the presence of organic matter, under constant influence of the sea. Usually, mangrove soils occur in regions of flat topography in the coastal strip. The soil of the transitional forest was a Neosoil composed by thin organic material or mineral material (EMBRAPA, 2013).
The climate is hot and temperate (Cfa in the Köeppen and Geiger classification), with average annual temperature around 21.1 °C and with 1 706 mm annual rainfall to the year of 2016 (Climate-Data, 2016). The sampling period (March/2016) corresponded to the end of the summer season, with average temperature of 24.1 oC and a mean precipitation of 204 mm.
Soil analysis: In both formations, we evaluated soil chemical characterization from one composed sample, collected 15 cm deep and homogenized, according to the methodology proposed by EMBRAPA (2013). We performed soil characterization using the Soil Analysis Laboratory (LAS) of the Agricultural Research and Rural Extension Company of Santa Catarina (Epagri), considering nutritional status and texture (EMBRAPA, 2013). We collected interstitial water using a sterile collecting device after digging holes into the soil of both study areas with the aid of a hollow tube. The salinity was measured using a refractomer (Vodex). The collections took place in March/2016.
Wood analysis: A total of ten wood samples - one sample per tree, from the outer wood region (close to the vascular cambium) - from mature populations, were taken at breast height (1.30 m high) from five individuals per population, during low tide following the Tides Tables (Epagri/Ciram, 2014), on the same period of March of 2016, which corresponded to the driest period of the year. Mature individuals were defined as adult trees which have been through reproductive periods. In the mangrove area, individuals were sampled near the fringe. Samples were registered in the Wood Library JOlw under the numbers JOlw 1 129 to 1 138 (collection number JCM 1 401 to 1 409 and 1 010) for mangrove samples and JOlw 1 119 to 1 128 (collection number JCM 1 411 to 1 419 and 1 020) for transitional mangroves samples.
Wood samples of 3 cm3 were used for histological preparation accordingly to standard techniques in wood anatomy (Johansen, 1940). We softened wood samples through cooking in glycerinated water 30 % (Ferreirinha, 1958). To obtain permanent histological slides, histological sections were made in transverse, tangential longitudinal and radial longitudinal sections planes (Johansen, 1940). Then, sections were clarified in sodium hypochlorite, washed in distilled water, stained with Safrablau (Bukatsch, 1972) and dehydrated in increasing ethyl series (Kraus & Arduim, 1997). We prepare slides using stained glass synthetic resin (Vasconcelos, MilanezeGutierre, & Inada, 2006).
Descriptions terminology followed the recommendations of the IAWA List of Microscopic Features for Harwood Identification (IAWA Committee, 1989). Thirty measurements of wood attributes were measured: tangential diameter (μm) and vessel frequency (n/mm²), vessel and fiber length (μm), fiber wall thickness (μm), height and width (number of cells and μm).
We calculated mean values and standard deviations for all anatomical attributes. We compared mean values by Student’s t-test, with a significance level of 5 %, in “R” (R Development Core Team, 2011) with the “labdsv” package (Roberts & Roberts, 2007), following the normality test and homogeneity of variance when necessary.
Results
Soil analysis: Mangrove soils showed higher values for porewater salinity (5.05 %) and higher pH (6.4), phosphorus (50 mg.dm-3), potassium (142 mmolc. dm-3), calcium (291 mmolc. dm-3) and sodium (685 mg.dm-3) contents, base sum (422.4 mmolc. dm-3) and base saturation percentage (96.46 %). The soil of the transitional forest presented lower porewater salinity (0.68 %) and pH (3), probably due to the higher amount of aluminum (6.3 mg.dm-3) and exchangeable cations (1 100 mmolc. dm-3), expressed by the cation exchange capacity (1 100 mmolc. dm-3), and the aluminum saturation (863.4 mmolc. dm-3; Table 1). Both areas presented the same amount of magnesium (97/95 mmolc. dm-3), organic matter (10 %) and boron (1.24/1.22 mg.dm-3).
Table 1 Soil properties (mean values, n=5) of mangrove and transitional forest at the Caieira Municipal Natural Park, Joinville, Santa Catarina, Brazil
Soil properties | Mangrove | Transitional |
---|---|---|
Porewater Salinity (%) | 5.05 | 0.68 |
Clay percentage (%) | 11 | 9 |
pH | 6.4 | 3 |
SMP index | 6.9 | 3.4 |
Phosphorus content (mg.dm-3) | 50 | 16.1 |
Potassium content (mmolc.dm-3) | 142 | 10 |
Organic matter (%) | 10 | 10 |
Aluminum content (mg.dm-3) | 0.3 | 6.3 |
Calcium content (mmolc.dm-3) | 291 | 122 |
Magnesium content (mmolc.dm-3) | 97 | 95 |
Sodium content (mg.dm-3) | 685 | 471 |
H+Al (mmolc.dm-3) | 15.5 | 863.4 |
Sum of bases (mmolc.dm-3) | 422.4 | 238.4 |
Cation exchange capacity (mmolc.dm-3) | 437.9 | 1 100 |
Bases saturation (%) | 96.46 | 21.64 |
Sulfur content (mg.dm-3) | 0.11 | 0.51 |
Boron content (mg.dm-3) | 1.24 | 1.22 |
Wood anatomical description (Figure 2): L. racemosa presented indistinct growth rings and diffuse porous wood, without specific vessel arrangement. Vessels were solitary and multiples of 2 to 3, rare 4-5, with simple perforation plate. Bordered intervessel pits were alternate; radio-vessel bordered pits were larger than the intervessel pits. Fibres had minute to simple bordered pits; layers of thin-walled fibres alternate with layers of thick-walled fibres. Axial paratracheal parenchyma was aliform lonsangular to confluent; starch grains were present in the axial parenchyma. Rays were heterogeneus and uniseriate, with body composed of procumbent cells, and margins of erect and squared cells.
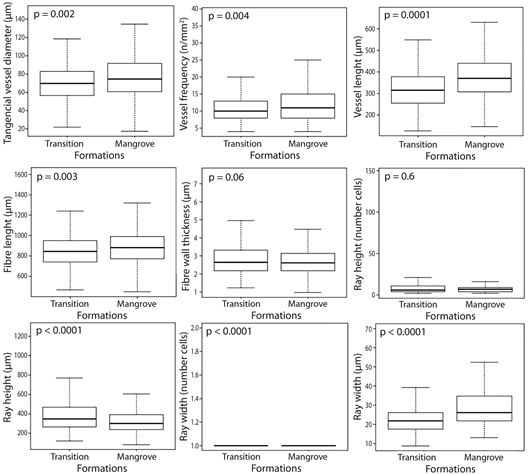
Figure 2 Comparative wood anatomy of Laguncularia racemosa (Combretaceae). A - B: cross section showing vessel diameter and vessel frequency in mangrove (A) and transitional forest (B). C - D: radial section showing height and width of rays in mangrove (C) and transitional forest (D). E: vessel length in mangrove (Mg) and transitional forest (Tr). F: fiber height in mangrove (Mg) and transitional forest (Tr). Scale bars = 100 μm.
Regarding quantitative data, populations of L. racemosa presented differences for all studied wood anatomical parameters, except for mean fiber wall thickness (Table 2, Figure 3). Mangrove population had the highest values for mean vessel diameter, length and frequency, ray width and fiber length. The transitional forest population had the mean higher rays. Mean fiber wall thickness was equal in both populations.
Table 2 Mean values and standard deviation of wood attributes of Laguncularia racemosa (Combretaceae) populations in mangrove and transitional forest populations of the Caieira Municipal Natural Park, Joinville, Santa Catarina, Brazil (n= 30)
Atributes | Mangrove | Transitional |
---|---|---|
Vessel diameter (µm) | 75.85 ± 22.86 ᵃ | 70.38 ± 20.90 ᵇ |
Vessel length (µm) | 375.79 ± 100.43 ᵃ | 317.52 ± 92.54 ᵇ |
Vessel frequency (n/mm²) | 11.90 ± 4.92 ᵃ | 10.87 ± 3.87 ᵇ |
Ray width (µm) | 29.38 ± 10.87 ᵃ | 21.73 ± 6.14 ᵇ |
Ray height (µm) | 338.56 ± 149.84 ᵇ | 392.80 ± 188.90 ᵃ |
Fibre length (µm) | 889.89 ± 171.06 ᵃ | 849.92 ± 166.35 ᵇ |
Fibre wall thickness (µm) | 2.70 ± 0.89 ᵃ | 2.84 ± 0.92 ᵃ |
Discussion
The higher variation of sodium content between the areas can be explained by the proximity to salty waters (Prada-Gamero, VidalTorrado, & Ferreira, 2004). Sodium is one of the cations associated to saline soils (Maksimovic & Ilin, 2012). The transitional forest area is not directly affected by salty water flooding, so its concentration of calcium was lower than in mangrove area. Another cation directly affected by salinity is calcium, which presents higher concentrations in more saline soils (Maksimovic & Ilin, 2012). In higher salinity soils with unconstrained sediments, calcium may act as a secondary messenger in cellular processes adjustments (Madi et al., 2015).
The porewater salinity, linked to tides dynamics, vary between areas closer to the salt water or to the restinga forest (Kathiresan & Bingham, 2001). Soils cation exchange capacity in mangroves is correlated to the flooding amplitude and tide duration (Ukpong, 2000; Naidoo, 2010; Urrego, Molina, & Suárez, 2014), resulting in salinity gradients and ions concentration along the flooding plateau. Both population samples were collected in the dry season, in low tides, and in the transitional forests floodings are less frequent and the influence of tides is low.
We found lower values of pH in the transitional area, which may influence the availability of nutrients (Ukpong, 2000; Krauss et al., 2008; Lima & Tognella, 2012). It is known that the pH changes the availability of important nutrients such as phosphorus, and non-essential like aluminium (Hossaim & Nuruddin, 2016). This situation was observed in the mangrove study area, where we found most of the nutrients in higher concentration and the pH is near to neutral. On the other hand, phosphorus is one nutrient highly sensitive to pH variation, in more acidic or alkaline soils, phosphorus tends to be less available. In this study, the contents of phosphorus in the transitional forests were much lower than in the mangroves, reflecting this situation.
Mangrove species are subject to extremely low water potential in the soil, because of high salinity, constraining hydraulic conductivity by the xylem (Lovelock et al., 2007). The presence of salt reduces the availability of water to the plant, known as physiological drought (Maksimovic & Ilin, 2012). This leads to a constant trade-off between safety against cavitation and efficient hydraulic conductivity (Sobrado, 2007; (Robert, Koedam, Beeckman, & Schmitz, 2009; Schmitz, Robert, Verheyden, Kairo, Beeckman, & Koedam, 2008). In order to minimize effects of cavitation due to a physiological drought, it is expected that plants present narrower and shorter vessel elements in higher densities (Sobrado, 2007; Schmitz et al., 2008). However, in the present study we found a higher vessel frequency of larger diameter in the higher salinity population (mangrove area), indicating that the individuals are investing more in hydraulic conduction than safety. Schmitz, Verheyden, Beeckman, Kairo, and Koedam (2006) demonstrated the effect of salinity in vessel frequency, the higher the salinity, the higher the frequency of vessels. The authors showed that vessel diameter was less affected by salinity. Yáñez-Espinosa, Terrazas, Lopez-Mata, and Valdez-Hernandez (2004) and Robert et al. (2009) observed the same relation between salinity and higher vessel frequency for Rhizophora mucronata Lam. (Rizophoraceae). Schmitz et al. (2006) suggested that a higher vessel frequency optimizes water transport under stressful conditions (high salinity), allowing the maintenance of functional and embolized vessels proportion, as showed in other studies (Baas et al., 1983; Mauseth & Plemons-Rodriguez, 1997).
Fiber wall thickness was also linked to water conduction, as a safety strategy in conditions of highly negative pressures within the secondary xylem, gave support to the vessels, and avoid collapse and cavitation (Hacke & Sperry, 2001; Jacobsen, Ewers, Pratt, Paddock III, & Davis, 2005).
Chimelo and Mattos-Filho (1988) observed wider rays in environments with larger water restrictions. Our results showed that the two populations have nearly the same amount of radial parenchyma, compensating the lower width with height and vice-versa. According to Tyree, Salleo, Nardini, Lo Gullo, and Mosca (1999), radial parenchyma is probably related to embolism repair, which is importantly fundamental for mangrove species subject to a constant physiological drought. Zheng and Martínez-Cabrera (2013) showed that rays size might vary in function: wider rays are more related to conductivity while narrower to mechanical strength.
This study showed that L. racemosa presents distinct wood anatomical features that demonstrate the plasticity of the tissue face to soil conditions, mainly those related to hydraulic conductivity (vessels). Possibly the population of transitional area is subject to a more unstable situation, although presenting less saline soils, the very low pH and nutrients availability are possible factors that affect xylem structure and conductivity.